Submitted by taire on
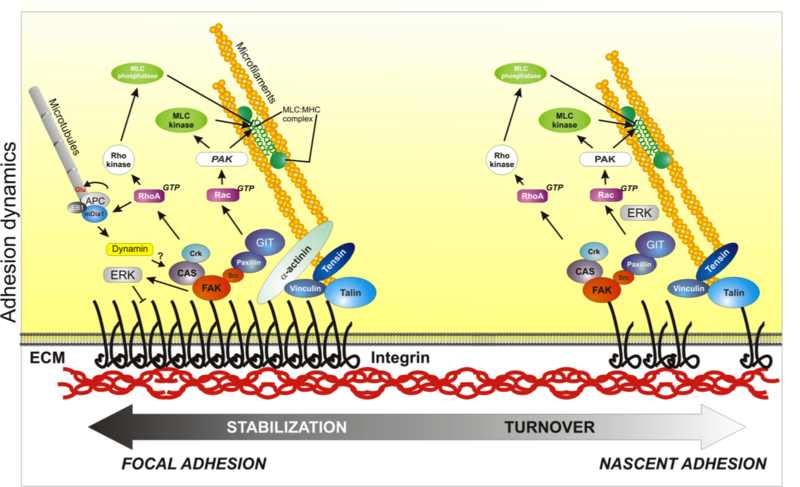
Scheme depicts the process of adhesion maturation. Nascent adhesions (on the right) are connected to the actin cytoskeleton by an actin linkage that includes talin, vinculin and α-actinin; other signaling adaptors are also recruited to these complexes, including FAK and paxillin. These adhesions generate signals that activate Rac, promoting actin polymerization and preventing myosin II engagement in the lamellipodium. These signals are also required for the dissolution of some adhesions (turnover) as the cell advances. Some adhesions mature into focal adhesions; this process is characterized by an enlargement and elongation of the complexes as well as the appearance of new players, like tensin. Some components have conformations that are tension sensitive (like talin, vinculin, and p130Cas, not shown here). Rho activation is thought to generate focal adhesions and actin bundling due to increased myosin II activity. Other events that occur at adhesions are internalization of the integrins, which is mediated by dynamin; and microtubule targeting, which may contribute to adhesion disassembly. Used with permission from Vicente-Manzanares et al., 2005 J.Cell Sci. 118,4,4917. click to enlarge
Cell adhesion (the physical interaction of a cell with another cell or with the extracellular matrix (ECM)) is essential for cell migration and tissue integrity. Cell-cell adhesion maintains epithelial tissues, supports functional contacts between specialized cells, and can facilitate directed migration (for example, radial glia can guide neuronal progenitor cells to specific layers of the brain). Cell-matrix adhesion is the best-studied form of adhesion that mediates cell migration, and is the focus of this outline.
Cell-matrix adhesions
Cell-matrix adhesions were first observed in cultured cells[1],[2],[3],[4]. They are sites of convergence between the actin cytoskeleton and ECM fibrils. Owing to their highly localized nature, cell-matrix adhesions were initially called focal adhesions. Over the years, several types of cell-matrix adhesion have been described.
- Nascent adhesions are the first observable adhesive structures, emerging within the lamellipodium. Their formation and stability are linked to the dendritic actin that forms[5],[6]. Nascent adhesions are small and highly transient - either maturing or disassembling ('turning over') - and are therefore not easily observed in every cell type[6],[7].
- Focal complexes are adhesions in the early stages of maturation. They were first observed in cells expressing a constitutively active form of Rac[8]. They are larger than nascent adhesions, depend on myosin II for their formation and maintenance, and reside at the boundary of the lamellum and lamellipodium[10],[11]. Like nascent adhesions, they also tend to either disassemble (turnover) or grow and elongate into focal adhesions. The elongation occurs along a template of bundled actin[7]. The presence of nascent adhesions and focal complexes is a marker of highly migratory cells; their fast appearance and turnover correlates directly with high velocities of protrusion and movement.
- Focal adhesions: this term is currently reserved for mature adhesions that evolve slowly over time[12]. These adhesions are usually physically linked to large, readily visualized actomyosin bundles[13], and their appearance correlates inversely with motility, such that they are conspicuously absent in cells migrating within a three-dimensional (3D) substrate. Although focal adhesions are similar, in terms of molecular composition, to nascent adhesions and focal complexes, several adhesion proteins appear to change as focal adhesions evolve in response to tension, e.g., zyxin and vinculin. Fibrillar adhesions represent an extreme in the maturation of adhesions and are not generally seen in migrating cells. They are characterized by the presence of tensin, large stress fibers, and underlying fibrillar fibronectin.
- Podosomes are ring-shaped adhesions often found in fast-moving cells, such as macrophages. Although their molecular composition is very similar to that of focal adhesions, their spatial distribution is radial, forming dot-like structures similar to 'suction cups'[14]. Podosomes are structurally divided into a core, which mainly contains proteins involved in actin polymerization (such as WASP, the Arp2/3 complex and cortactin), and a surrounding ring populated by integrin receptors and adhesion proteins (for example, paxillin and focal adhesion kinase (FAK/Pyk2))[15]. Whereas the formation of focal adhesions requires actomyosin-mediated contractile force mediated by RhoA, podosome formation is stimulated by a local loss of contractility and the recruitment of negative regulators of RhoA, such as p190RhoGAP[16].
- Invadopodia are specialized actin-based structures that are very similar morphologically to podosomes, but are often found in invading cells, such as metastatic tumor cells[14],[17]; the consensus seems to be to use 'podosome' to apply to normal cells and 'invadopodium' for cancer cells. Like podosomes, invadopodia are mainly radial; however, adhesion proteins are not restricted to the peripheral ring, but appear intermixed with the structural core, where most actin-related proteins reside[18]. In addition to their adhesive nature, invadopodia often concentrate proteolytic components that degrade the surrounding matrix, to permit eventual migration of the cell through the matrix [19].
Important components of cell-matrix adhesions
The different types of matrix adhesion share many common components, as outlined below.
Integrins: Adhesion receptors constitute the physical link between the cell and the ECM. The best-studied adhesion receptors are type I transmembrane proteins that comprise the integrin family. Many combinations of the α and β subunits exist to give rise to multiple heterodimers that interact with specific ligands - components of the ECM[20],[21]. Integrins are finely regulated receptors; some are designed to engage in only appropriate circumstances (for example, to mediate platelet or leukocyte adhesion).
Integrins modulate the adhesiveness of a cell to the ECM in several ways. One way is by forming large clusters that increase the avidity of binding. The formation of clusters is usually driven by molecules that bind to the cytoplasmic tails of the integrins and crosslink them[22]. In some cases, the presence of membrane microdomains such as cholesterol-enriched domains and other lipid rafts or other transmembrane proteins (for example, tetraspanins) might modulate the avidity of binding[23],[24],[25]. Another way through which integrins modulate adhesion is through a conformational change that creates a high-affinity state[26]. The conformational changes are induced by the binding of molecules such as talin or kindlin to the intracellular portion of the integrin receptor subunit, which causes the molecule to extend and expose the sites of ligand binding ('affinity modulation', induced by so-called inside-out signaling)[26],[27],[28],[29]. Talin, in turn, undergoes conformational extension in response to other stimuli through a RAP1-RIAM (Rap1-interacting adaptor molecule) pathway, which is regulated by growth factors[30]. Integrins can also initiate intracellular signals following ligation by either direct or indirect association of adaptor and other signaling molecules with their cytoplasmic tails, a process termed 'outside-in' signaling.
Actin-ECM linkage: Some of the proteins that bind to the cytoplasmic tail of integrins also bind directly or indirectly to actin. These proteins include talin, vinculin and α-actinin. Notably, vinculin not only binds to actin, but also to the Arp2/3 complex, which nucleates actin filaments in the lamellipodium[31]. Talin binds to both integrin and actin[32],[33]; vinculin binds to talin and activates it[34],[35],[36]. α-actinin, which binds to actin, is also reported to bind β1 integrins directly[37] and also indirectly through vinculin[38]. Another crosslinking protein, filamin, also binds to the cytoplasmic tails of β1 and β7 integrins[39].
These linkages define a molecular linkage, or clutch, that shunts retrograde forces from actomyosin contraction or resistance generated by the leading edge membrane to the ECM. The efficiency of the clutch - that is, the degree of engagement (binding strength) between the components of the linkage (ECM proteins, integrins, actin-binding adaptors and the actin filaments) - regulates the coupling between adhesion and protrusion.
Talin, vinculin and α-actinin can all regulate integrin avidity by clustering integrins and actin filaments. The binding strength of these proteins to integrin and/or actin is tightly regulated. Mechanisms of regulation include conformational changes induced by binding to their partners (for example, vinculin to talin or actin[36]), phosphorylation (for example, α-actinin[40]), or mechanical force (for example, talin[41],[42]). There is also evidence that integrins themselves modulate their activation in response to mechanical forces[43].
Signaling: A plethora of signaling molecules also associate with integrin-containing adhesions. Some are kinases, whereas others function as scaffolds that recruit additional molecules to adhesions. A key signature of several adhesion proteins is their prominent phosphorylation on tyrosine residues[44].
- The tyrosine kinase FAK is recruited to adhesions early and is activated by autophosphorylation and Src-family kinases[45]. The FAK-Src module is implicated in the disassembly of adhesion complexes[46]. FAK recruits and, in conjunction with Src, phosphorylates other adhesion proteins, like paxillin, and potentially controls RhoA activation by binding its GEFs and GAPs. For example, FAK binds p190RhoGEF (a RhoA activator) and p190RhoGAP (a RhoA inhibitor) via p120RasGAP[47]; the spatiotemporal regulation of these interactions likely determines the local activation of the GTPase.
- Paxillin is a phosphoprotein that localizes to adhesions. Through multiple phosphorylated tyrosine and serine/threonine residues, it creates binding sites that recruit other adhesion proteins and signaling regulators. Although paxillin does not bind Rac directly, it is emerging as a signaling hub for Rac, due to its ability to recruit molecules (mainly GEFs) implicated in activating Rac.
- p130 Crk-associated substrate (p130Cas) is a phosphoprotein that localizes to adhesions by binding to paxillin and other molecules, such as FAK or Nck[48]. It is implicated in the recruitment and activation of DOCK180, a Rac GEF, throughCrk to adhesions, and in the activation of Rac[49]. p130Cas responds to mechanical force, becoming phosphorylated in response to stretching[50].
- Zyxin is a scaffold protein that is distantly related to paxillin. It interacts with α-actinin and might be involved in regulating actin polymerization near adhesions via its association with VASP[51]. Zyxin is also mechanoreactive, shuttling between actin/α-actinin and adhesions in response to stretching[52].
- Tensin is a phosphoprotein that is present only in very large, mature adhesions[8], and has a prominent role in signaling through its interaction with protein phosphatases[53].
The proteins outlined above are only a sample of the best-characterized adhesion molecules; more than 180 proteins participate in a complicated network of interactions called the 'adhesome'[54],[55]. The components of adhesions and their interactions depend on the type of adhesion, the integrins that are present, the presence of other receptors, and the cell type. These complicated signaling networks generate a plethora of signals that not only control cell migration (by controlling actin polymerization and disassembly, adhesion formation and assembly, etc.), but also many other cellular functions, including proliferation, gene expression and cell survival. Furthermore, adhesion is central in the cell's ability to sense its surroundings. Adhesive foci act as the cell's 'sensory organs', which inform the cell of the mechanical and chemical properties of its environment. These data are integrated by the different signaling networks, generating polarized responses that control protrusion, formation of new adhesions at the front and disassembly at the rear of the cell.
- Curtis, A. S. The Mechanism of Adhesion of Cells to Glass. A Study by Interference Reflection Microscopy. J Cell Biol 20, 199-215 (1964). Pubmed abstract # 14126869
- Lazarides, E. & Burridge, K. Alpha-actinin: immunofluorescent localization of a muscle structural protein in nonmuscle cells. Cell 6, 289-98 (1975). Pubmed abstract # 802682
- Izzard, C. S. & Lochner, L. R. Cell-to-substrate contacts in living fibroblasts: an interference reflexion study with an evaluation of the technique. J Cell Sci 21, 129-59 (1976). Pubmed abstract # 932106
- Heath, J. P. & Dunn, G. A. Cell to substratum contacts of chick fibroblasts and their relation to the microfilament system. A correlated interference-reflexion and high-voltage electron-microscope study. J Cell Sci 29, 197-212 (1978). Pubmed abstract # 564353
- Geiger, B. A 130K protein from chicken gizzard: its localization at the termini of microfilament bundles in cultured chicken cells. Cell 18, 193-205 (1979). Pubmed abstract # 574428
- Alexandrova, A. Y. et al. Comparative dynamics of retrograde actin flow and focal adhesions: formation of nascent adhesions triggers transition from fast to slow flow. PLoS One 3, e3234 (2008). Pubmed abstract # 18800171
- Choi, C. K. et al. Actin and alpha-actinin orchestrate the assembly and maturation of nascent adhesions in a myosin II motor-independent manner. Nat Cell Biol 10, 1039-50 (2008). Pubmed abstract # 19160484
- Zaidel-Bar, R., Ballestrem, C., Kam, Z. & Geiger, B. Early molecular events in the assembly of matrix adhesions at the leading edge of migrating cells. J Cell Sci 116, 4605-13 (2003). Pubmed abstract # 14576354
- Ridley, A. J., Paterson, H. F., Johnston, C. L., Diekmann, D. & Hall, A. The small GTP-binding protein rac regulates growth factor-induced membrane ruffling. Cell 70, 401-10 (1992). Pubmed abstract # 1643658
- Giannone, G. et al. Lamellipodial actin mechanically links myosin activity with adhesion-site formation. Cell 128, 561-75 (2007). Pubmed abstract # 17289574
- Rottner, K., Hall, A. & Small, J. V. Interplay between Rac and Rho in the control of substrate contact dynamics. Current Biology 9, 640-649 (1999). Pubmed abstract # 10375527
- Zaidel-Bar, R., Cohen, M., Addadi, L. & Geiger, B. Hierarchical assembly of cell-matrix adhesion complexes. Biochem Soc Trans 32, 416-20 (2004). Pubmed abstract # 15157150
- Hotulainen, P. & Lappalainen, P. Stress fibers are generated by two distinct actin assembly mechanisms in motile cells. J Cell Biol 173, 383-94 (2006). Pubmed abstract # 16651381
- Gimona, M., Buccione, R., Courtneidge, S. A. & Linder, S. Assembly and biological role of podosomes and invadopodia. Curr Opin Cell Biol 20, 235-41 (2008). Pubmed abstract # 18337078
- Buccione, R., Orth, J. D. & McNiven, M. A. Foot and mouth: podosomes, invadopodia and circular dorsal ruffles. Nat Rev Mol Cell Biol 5, 647-57 (2004). Pubmed abstract # 15366708
- Lener, T., Burgstaller, G., Crimaldi, L., Lach, S. & Gimona, M. Matrix-degrading podosomes in smooth muscle cells. Eur J Cell Biol 85, 183-9 (2006). Pubmed abstract # 16546560
- Linder, S. Invadosomes at a glance. J Cell Sci 122, 3009-13 (2009). Pubmed abstract # 19692587
- Block, M. R. et al. Podosome-type adhesions and focal adhesions, so alike yet so different. Eur J Cell Biol 87, 491-506 (2008). Pubmed abstract # 18417250
- Wolf, K. & Friedl, P. Mapping proteolytic cancer cell-extracellular matrix interfaces. Clin Exp Metastasis 26 289-98 (2009).Pubmed abstract # 18600304
- Hynes, R. O. Integrins: bidirectional, allosteric signaling machines. Cell 110, 673-87 (2002). Pubmed abstract # 12297042
- Humphries, J. D., Byron, A. & Humphries, M. J. Integrin ligands at a glance. J Cell Sci 119, 3901-3 (2006). Pubmed abstract # 16988024
- Carman, C. V. & Springer, T. A. Integrin avidity regulation: are changes in affinity and conformation underemphasized? Curr Opin Cell Biol 15, 547-56 (2003). Pubmed abstract # 14519389
- Krauss, K. & Altevogt, P. Integrin leukocyte function-associated antigen-1-mediated cell binding can be activated by clustering of membrane rafts. J Biol Chem 274, 36921-7 (1999). Pubmed abstract # 10601245
- Hemler, M. E. Tetraspanin functions and associated microdomains. Nat Rev Mol Cell Biol 6, 801-11 (2005). Pubmed abstract # 16314869
- Yanez-Mo, M., Barreiro, O., Gordon-Alonso, M., Sala-Valdes, M. & Sanchez-Madrid, F. Tetraspanin-enriched microdomains: a functional unit in cell plasma membranes. Trends Cell Biol 19, 434-46 (2009). Pubmed abstract # 19709882
- Shattil, S. J., Kim, C. & Ginsberg, M. H. The final steps of integrin activation: the end game. Nat Rev Mol Cell Biol 11, 288-300 (2010). Pubmed abstract # 20308986
- Kim, M., Carman, C. V. & Springer, T. A. Bidirectional transmembrane signaling by cytoplasmic domain separation in integrins. Science 301, 1720-5 (2003). Pubmed abstract # 14500982
- Ye, F. et al. Recreation of the terminal events in physiological integrin activation. J Cell Biol 188, 157-73 (2010). Pubmed abstract # 20048261
- Askari, J. A. et al. Focal adhesions are sites of integrin extension. J Cell Biol 188, 891-903 (2010). Pubmed abstract # 20231384
- Watanabe, N. et al. Mechanisms and consequences of agonist-induced talin recruitment to platelet integrin alphaIIbbeta3. J Cell Biol 181, 1211-22 (2008). Pubmed abstract # 18573917
- DeMali, K. A., Barlow, C. A. & Burridge, K. Recruitment of the Arp2/3 complex to vinculin: coupling membrane protrusion to matrix adhesion. J Cell Biol 159, 881-91 (2002). Pubmed abstract # 12473693
- Horwitz, A., Duggan, K., Buck, C., Beckerle, M. C. & Burridge, K. Interaction of plasma membrane fibronectin receptor with talin--a transmembrane linkage. Nature 320, 531-3 (1986). Pubmed abstract # 2938015
- Critchley, D. R. Biochemical and structural properties of the integrin-associated cytoskeletal protein talin. Annu Rev Biophys 38, 235-54 (2009). Pubmed abstract # 19416068
- Burridge, K. & Mangeat, P. An interaction between vinculin and talin. Nature 308, 744-6 (1984). Pubmed abstract # 6425696
- Hemmings, L. et al. Talin contains three actin-binding sites each of which is adjacent to a vinculin-binding site. J Cell Sci 109 ( Pt 11), 2715-26 (1996). Pubmed abstract # 8937989
- Bakolitsa, C. et al. Structural basis for vinculin activation at sites of cell adhesion. Nature 430, 583-6 (2004). Pubmed abstract # 15195105
- Otey, C. A., Pavalko, F. M. & Burridge, K. An interaction between alpha-actinin and the beta 1 integrin subunit in vitro. J Cell Biol 111, 721-9 (1990). Pubmed abstract # 2116421
- Gilmore, A. P. & Burridge, K. Regulation of vinculin binding to talin and actin by phosphatidyl-inositol-4-5-bisphosphate. Nature 381, 531-5 (1996). Pubmed abstract # 8632828
- Pfaff, M., Liu, S., Erle, D. J. & Ginsberg, M. H. Integrin beta cytoplasmic domains differentially bind to cytoskeletal proteins. J Biol Chem 273, 6104-9 (1998). Pubmed abstract # 9497328
- Izaguirre, G. et al. The cytoskeletal/non-muscle isoform of alpha-actinin is phosphorylated on its actin-binding domain by the focal adhesion kinase. J Biol Chem 276, 28676-85 (2001). Pubmed abstract # 11369769
- Jiang, G., Giannone, G., Critchley, D. R., Fukumoto, E. & Sheetz, M. P. Two-piconewton slip bond between fibronectin and the cytoskeleton depends on talin. Nature 424, 334-7 (2003). Pubmed abstract # 12867986
- del Rio, A. et al. Stretching single talin rod molecules activates vinculin binding. Science 323, 638-41 (2009). Pubmed abstract # 19179532
- Friedland, J. C., Lee, M. H. & Boettiger, D. Mechanically activated integrin switch controls alpha5beta1 function. Science 323, 642-4 (2009). Pubmed abstract # 19179533
- Ballestrem, C. et al. Molecular mapping of tyrosine-phosphorylated proteins in focal adhesions using fluorescence resonance energy transfer. J Cell Sci 119, 866-75 (2006). Pubmed abstract # 16478788
- Parsons, J. T. Focal adhesion kinase: the first ten years. J Cell Sci 116, 1409-16 (2003). Pubmed abstract # 12640026
- Webb, D. J. et al. FAK-Src signalling through paxillin, ERK and MLCK regulates adhesion disassembly. Nat Cell Biol 6, 154-61 (2004). Pubmed abstract # 14743221
- Tomar, A. & Schlaepfer, D. D. Focal adhesion kinase: switching between GAPs and GEFs in the regulation of cell motility. Curr Opin Cell Biol 21, 676-83 (2009). Pubmed abstract # 19525103
- Defilippi, P., Di Stefano, P. & Cabodi, S. p130Cas: a versatile scaffold in signaling networks. Trends Cell Biol 16, 257-63 (2006). Pubmed abstract # 16581250
- Matsuda, M. et al. Interaction between the amino-terminal SH3 domain of CRK and its natural target proteins. J Biol Chem 271, 14468-72 (1996). Pubmed abstract # 8662907
- Sawada, Y. et al. Force sensing by mechanical extension of the Src family kinase substrate p130Cas. Cell 127, 1015-26 (2006). Pubmed abstract # 17129785
- Drees, B. E., Andrews, K. M. & Beckerle, M. C. Molecular dissection of zyxin function reveals its involvement in cell motility. J Cell Biol 147, 1549-60 (1999). Pubmed abstract # 10613911
- Yoshigi, M., Hoffman, L. M., Jensen, C. C., Yost, H. J. & Beckerle, M. C. Mechanical force mobilizes zyxin from focal adhesions to actin filaments and regulates cytoskeletal reinforcement. J Cell Biol 171, 209-15 (2005). Pubmed abstract # 16247023
- Hall, E. H., Daugherty, A. E., Choi, C. K., Horwitz, A. F. & Brautigan, D. L. Tensin1 requires protein phosphatase-1alpha in addition to RhoGAP DLC-1 to control cell polarization, migration, and invasion. J Biol Chem 284, 34713-22 (2009).Pubmed abstract # 19826001
- Zaidel-Bar, R., Itzkovitz, S., Ma'ayan, A., Iyengar, R. & Geiger, B. Functional atlas of the integrin adhesome. Nat Cell Biol 9, 858-867 (2007). Pubmed abstract # 17671451
- Zaidel-Bar, R. & Geiger, B. The switchable integrin adhesome. J Cell Sci 123, 1385-8 (2010). Pubmed abstract # 20410370