Submitted by taire on
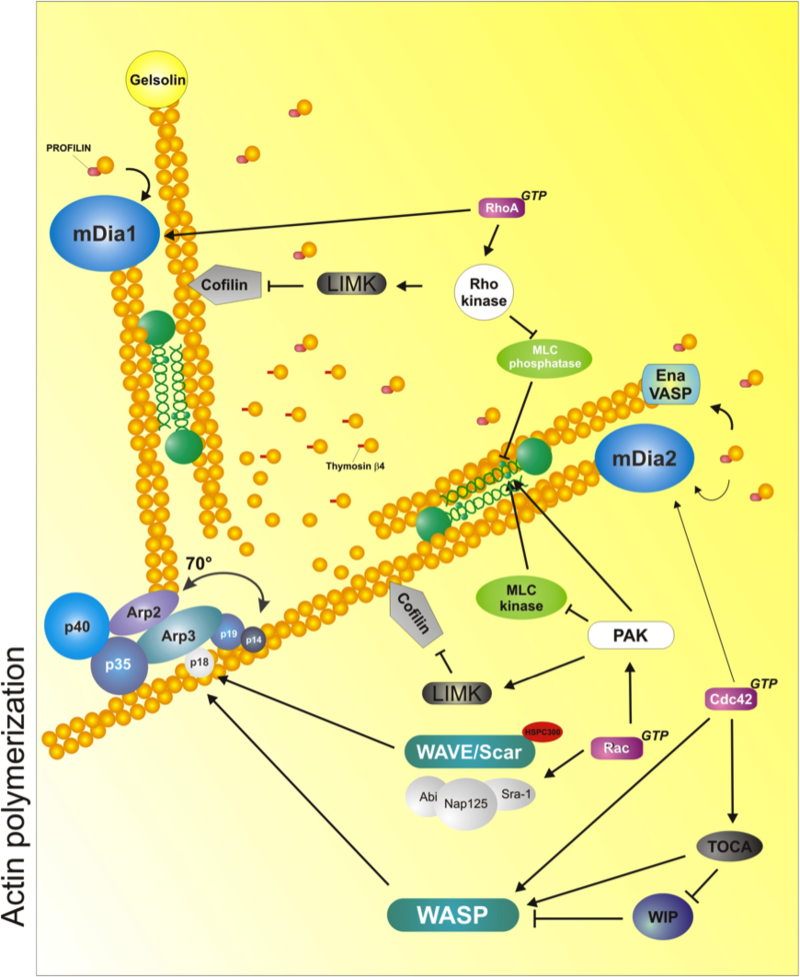
Scheme depicts the major mechanisms and molecules implicated in regulating and enabling actin polymerization/depolymerization and organization. The Arp2/3 complex induces branching by attaching to the side of a pre-existing filament and promoting de novo polymerization. Formins such as mDia1 and mDia2 induce processive actin polymerization by attaching themselves to the barbed end of the filament. The formins compete with actin capping proteins, such as gelsolin and capZ (not depicted). Ena/VASP serves a dual role as an adaptor protein and a 'leaky' capper, which enables actin polymerization through other molecules that are part of a complex (not shown). Myosin II (in green, between filaments) cross links antiparallel actin mediates contraction due to its ATPase-dependent motor domain, and its cross-linking, ATPase-independent activities. Cofilin (grey arrowhead) severs actin filaments, promoting the formation of new barbed ends. It also generates actin monomers to be incorporated to the filaments via profilin/formin (treadmilling). Another small protein, thymosin β4, sequesters actin monomers and maintains the pool of available monomers. Some of the signaling proteins that control these molecules are also shown. They include: Rho-kinase (ROCK), which is under the control of RhoA and activates LIMK to activate cofilin, blocks the myosin phosphatase, promoting myosin activation, and phosphorylates and activates myosin directly; PAK also activates LIMK but it inhibits MLCK, inactivating myosin II; the WAVE/Scar-Abi complex and WASP, which activate the Arp2/3 complex, are under the control of Rac and Cdc42, respectively; finally, formins are also activated by small GTPases directly (mDia1 by RhoA; mDia2 by Cdc42). Used with permission from Vicente-Manzanares et al., 2005 J.Cell Sci. 118,4,4917.
click to enlarge
Protrusion involves the extension of cellular membranes. In most cells, a leading protrusion points in the direction of movement and is part of a polarity axis (see section on Polarity). Protrusion usually occurs in response to chemoattractive signals in the microenvironment that are detected by the cell: for example, migrating amoebae respond to the feeding cue cyclic AMP, whereas leukocytes are induced to move towards pro-inflammatory cytokines released by injured tissue. However, some cells extend protrusions in a probing, exploratory manner in the absence of directional stimulation[1].
The formation of protrusions requires the integration of several cellular processes. The force for membrane deformation is provided by the actin cytoskeleton, the dynamic polymerization of which pushes the membrane forward. The membrane itself expands under tension, a process that requires membrane trafficking and the fusion of membrane-containing vesicles to support the increase in membrane surface. Finally, protrusions must adhere to the substrate. If they do not attach, protrusions are unproductive and tend to move rearward in waves in response to the tension generated in the cell, in a process known as 'membrane ruffling'.
The actin cytoskeleton
Actin filaments constitute the physical backbone of a protrusion. Their polymerization and organization also determine the overall shape of the cell and contribute to its internal organization. Actin filaments comprise arrays of monomeric globular (G)-actin subunits that polymerize in a process catalysed by ATP hydrolysis. During the polymerization process, ATP bound to G-actin is hydrolyzed to ADP⋅Pi as the bond between the two monomers forms. In filamentous (F)-actin, ADP⋅Pi-actin forms stable filaments; release of the phosphate destabilizes the structure, prompting depolymerization[2],[3],[4].
Similar to microtubules, actin filaments are intrinsically polarized, reflecting the oriented, head-to-tail structure of the constituent monomers, and display a fast-growing 'barbed' end, where new monomers are incorporated, and a 'pointed' end, which represents the origin of the growing filament and exhibits filament ageing (bound to ADP alone)[3],[4].
Actin filaments adopt different morphologies depending on the number of filaments and the type and number of actin-binding proteins that associate with them. Actin-binding proteins, such as myosins, α-actinins, filamins and others, have cross-linking activity, and can organize actin filaments into bundles or a branched network. If two filaments display their barbed ends in the same direction, the resulting bundle is parallel; if the barbed end and the pointed end point in opposite directions, the bundle is anti-parallel. The consequent organization of actin filaments into bundles or a branched network can give rise to different cellular structures.
Actin filaments adopt different morphologies depending on the number of filaments and the type and number of actin-binding proteins that associate with the filaments; the usual results are filament bundles or a branched, dendritic structure. The details of these associations lead to different cellular structures:
- Filopodia are long, thin protrusions that emerge from the cellular membrane. They are mainly regulated by the small Rho GTPase Cdc42 (see Rho GTPase box). They are made of long, unbranched, parallel actin bundles, often decorated with tropomyosin and fascin; their elongation is mediated by formins[5]. Filopodia carry out an exploratory function, enabling the cell to probe its local environment[6],[7].
- Lamellipodia are broad, sheet-like protrusions that contain a branched network of thin, short actin filaments [8],[9]. In a now-classic view, lamellipodia are generated by the small GTPase Rac and some of its effectors, notably the WAVE/Scar complex and N-WASP, which control Arp2/3 (see boxes on small Rho GTPases and actin polymerization, respectively). Behind a lamellipodium there is often another structure, the lamellum, in which actin is bundled rather than branched[8],[9],[10]. Most motile protrusions display a thin (~1 μm) lamellipodium closer to the membrane, followed by a wider lamellum (5-10 μm) closer to the nucleus. Some uncertainty exists about the dendritic structure of lamellipodia and their relationship with lamellae[10],[11],[12],[13].
- Stress fibers are thick bundles of actin filaments that contain many anti-parallel actin filaments. The filaments are heavily decorated with myosin II, which endows them with contractile properties[14]. The small Rho GTPase RhoA is a key regulator of stress fiber formation (see Rho GTPases box). Stress fibers are often found along the ventral portion of the cell and terminate in a large adhesive structure known as a focal adhesion at one end[15].
- Arches (dorsal or ventral) are long, thick bundles of actin filaments that provide transverse structural support for the cell. They are tethered to the dorsal and side portions of the cell and sometimes terminate in adhesions[15].
Box: Regulation of actin polymerization
Elongation of parallel bundles of filaments in filopodia and of dendritic networks leading to sheet-like protrusions comprising lamellipodia and lamellae is actively driven by actin polymerization, which is regulated by multiple regulatory proteins.
Proteins that bind to G actin: Two molecules regulate the availability of actin monomers for polymerization - profilin and thymosin β4. Both molecules bind specifically to G-actin, but profilin can also bind to different actin polymerization nucleators and stimulators (e.g. formins; see below), and thereby functions as an actin monomer 'shuttle'[16]. By contrast, as thymosin β4 does not bind to any nucleator, it is believed to sequester G-actin and maintain an appropriate reservoir that enables the release of G-actin to profilin to promote the growth of actin filaments[3],[4],[16],[17].
Proteins that maintain the pool of G-actin: Monomeric actin is mainly derived from two sources. One is de novo synthesis. Notably, actin synthesis is concentrated at sites of protrusion, as revealed by the presence of β-actin messenger RNA in protrusions[18]. Monomer recycling, driven by depolymerization of preformed filamentous structures, provides the other source. Depolymerization is a spontaneous process that occurs at the pointed ends owing to filament ageing (bound to ADP alone; see above), and also owing to filament severing, which is catalysed by ADF (ADP depolymerization factor)/cofilin. As a consequence of its severing function, cofilin carries out a dual purpose: creating new free ends from which polymerization can be reinitiated, and also breaking down and depolymerizing old networks for monomer recycling[19],[20]. Cofilin is regulated by phosphorylation by LIM kinase (LIMK), which is, in turn, regulated by phosphorylation by p21-activated kinase (PAK) or Rho-kinase (ROCK)[20],[21]. There is also evidence that another actin-binding protein, coronin, regulates cofilin activity through recruitment of a specific phosphatase[22]. Coronin, in contrast to cofilin, binds to the sides of actin filaments and protects them from cofilin-mediated severing and depolymerization[23].
Proteins that mediate actin polymerization at the barbed end: Polymerization occurs at the barbed end of actin filaments much more efficiently than at the pointed end. This process is regulated by several proteins, which can be divided into two antagonistic groups: barbed-end polymerization promoters and capping proteins[24].
Promoters include direct nucleators - for example, formins[25]. Formins are multi-modular proteins that bind to the barbed end of actin, to profilin and, in some cases, to microtubules, regulating their stability. They function as processive polymerization devices that initiate de novo actin filament assembly by recruiting actin monomers and using them to nucleate new filaments. Formins are then able to maintain filament elongation by remaining bound to the fast-growing end and promoting addition of further monomers, simultaneously preventing binding of capping proteins (see below)[25],[26]. There are many formins, the best characterized of which are mDia1, mDia2 and mDia3. Their activity is regulated by binding of small Rho GTPases (for example, RhoA regulates mDia1 and Cdc42 regulates mDia2), which relieves head-to-tail autoinhibitory interactions in the formins[26]. Other proteins, such as vasodilator stimulated phosphoprotein (VASP) and its relatives Mena and Ena/VASP-like (EVL) also prevent binding of capping proteins and promote actin polymerization through profilin, but cannot nucleate filaments de novo[27],[28].
Capping proteins terminate elongation, thereby limiting polymerization of new filaments[29]. CapZ is an α/βheterodimeric capping protein that is found in most eukaryotic cells, but there are others, such as adducin, which caps the barbed end of short actin filaments. Perhaps the best known capping protein is gelsolin/brevin, which is one of a series of family members that includes capG, severin, villin, adseverin, advillin and supervillin[30]. These proteins bind to the barbed ends with high affinity in the presence of calcium, and are also regulated by phosphoinositides[30].
Proteins that regulate actin nucleation at the pointed end: In lamellipodia, actin nucleators that bind to the pointed end and promote polymerization can provide a scaffold to support polymerization at the barbed end. This role is mainly carried out by a complex of actin-related proteins 2 and 3 (the Arp2/3 complex), a heteroheptamer that binds to the side of a preformed actin filament and promotes actin polymerization, forming a 70° angle with the pre-existing filament[31]. This binding and subsequent polymerization imposes a branched geometry on the actin network.
The Arp2/3 complex is activated locally at the cell membrane by proteins of the WASP/WAVE family. The WASP family contains two main members: Wiskott-Aldrich syndrome protein (WASP), which is expressed exclusively in haematopoietic cells[32], and neuronal (N)-WASP, which - contrary to its namesake - is more widely expressed. Studies carried out within the past 5 years suggest that, although both Arp2 and Arp3 are required for nucleation in the presence of WASP, the loss of Arp2 does not interfere with binding to the N-WASP tail[33]. WASP/N-WASP/WAVE regulation of the Arp2/3 complex is tightly controlled by phosphorylation (WASP), small Rho GTPases (Cdc42 regulates WASP and N-WASP, Rac regulates the WAVE/Scar complex) and phosphoinositides[24],[32],[34]. The WAVE/Scar complex, (which includes the components WAVE, Abi, Nap125, Sra-1 and HSPC-300) is activated by binding of active Rac, which induces the dissociation of the trimer Abi-Nap125-Sra-1 from WAVE[35]. Additionally, Abi has been shown to connect the WAVE/Scar complex with formins[36], implying that the formation of the two distinct actin protrusions - Arp2/3-complex-generated branched networks and filopodia - may be linked[37].
Actin reorganization and bundling in the lamellum: In the lamellum, actin reorganization, rather than polymerization, is the leading mechanism thought to create the architecture in this region. Actin filaments are organized and bundled together in thick, linear, and mostly anti-parallel, arrays by the action of two families of actin-binding proteins: α-actinins and class II myosins. By crosslinking actin, these molecules generate supramolecular aggregates that bind simultaneously and bring together multiple individual actin filaments into stable bundles[17]. Myosin II also provides contractile force through an ATPase-driven conformational change that induces filament sliding[38]. In motile cells, myosin II activity undergoes regulation mainly by phosphorylation on its regulatory light chain by kinases that are, in turn, regulated by Rho GTPases, particularly RhoA. Other actin-binding proteins with crosslinking function - particularly tropomyosins and filamins - are also implicated in the creation of actin bundles in this region.
Membrane extension during protrusion
The membrane that forms the new protrusion could arise from the flow of membrane from other regions of the cell or trafficking of vesicles. Endocytic vesicles are targeted to the front and regulate actin polymerization through the localized delivery of pre-activated Rac, which depends on Rab5[39]. On the other hand, polarized exocytic delivery to the leading edge is a source of membrane that depends on microtubules and Rab8[40],[41]. Finally, protrusions are also sites of active endocytosis, which is important in the recycling of integrins and other receptors[42],[43].
Other modes of protrusion
In some specific cases, protrusion seems to occur in the absence of actin assemblies. For example, cells invading a three-dimensional (3D) matrix generate protrusive 'blebs', which are driven initially by hydrostatic pressure from inside the cell[44]. In this model, myosin II-based-contraction is thought to provide the contractile power that generates the hydrostatic extension of the blebs[45],[46]. Actin assembly is implicated in the later stages of protrusion to provide stability to the blebs (filling the bleb, which then evolves into a pseudopod/lobopod), or to retract it back into the cell membrane.
In summary, protrusion results from active actin polymerization and reorganization, and targeted membrane extension. Adhesion plays key roles in protrusion: it not only provides traction for membrane ruffles to become stable, but also nucleates and regulates signaling components that control actin reorganization and membrane endocytosis and delivery.
- Petrie, R. J., Doyle, A. D. & Yamada, K. M. Random versus directionally persistent cell migration. Nat Rev Mol Cell Biol 10 538-49 (2009). Pubmed abstract # 19603038
- Korn, E. D., Carlier, M. F. & Pantaloni, D. Actin polymerization and ATP hydrolysis. Science 238, 638-44 (1987). Pubmed abstract # 3672117
- Pollard, T. D. & Borisy, G. G. Cellular motility driven by assembly and disassembly of actin filaments. Cell 112, 453-65 (2003). Pubmed abstract # 12600310
- Pollard, T. D. & Cooper, J. A. Actin, a central player in cell shape and movement. Science 326, 1208-12 (2009). Pubmed abstract # 19965462
- Block, J. et al. Filopodia formation induced by active mDia2/Drf3. J Microsc 231, 506-17 (2008). Pubmed abstract # 18755006
- Mattila, P. K. & Lappalainen, P. Filopodia: molecular architecture and cellular functions. Nat Rev Mol Cell Biol 9, 446-54 (2008). Pubmed abstract # 18464790
- Mejillano, M. R. et al. Lamellipodial versus filopodial mode of the actin nanomachinery: pivotal role of the filament barbed end. Cell 118, 363-73 (2004). Pubmed abstract # 15294161
- Cramer, L. P., Siebert, M. & Mitchison, T. J. Identification of novel graded polarity actin filament bundles in locomoting heart fibroblasts: implications for the generation of motile force. J Cell Biol 136, 1287-305 (1997). Pubmed abstract # 9087444
- Svitkina, T. M. & Borisy, G. G. Arp2/3 complex and actin depolymerizing factor/cofilin in dendritic organization and treadmilling of actin filament array in lamellipodia. J Cell Biol 145, 1009-26 (1999). Pubmed abstract # 10352018
- Ponti, A., Machacek, M., Gupton, S. L., Waterman-Storer, C. M. & Danuser, G. Two distinct actin networks drive the protrusion of migrating cells. Science 305, 1782-6 (2004). Pubmed abstract # 15375270
- Koestler, S. A., Auinger, S., Vinzenz, M., Rottner, K. & Small, J. V. Differentially oriented populations of actin filaments generated in lamellipodia collaborate in pushing and pausing at the cell front. Nat Cell Biol 10, 306-13 (2008). Pubmed abstract # 18278037
- Vallotton, P. & Small, J. V. Shifting views on the leading role of the lamellipodium in cell migration: speckle tracking revisited. J Cell Sci 122, 1955-8 (2009). Pubmed abstract # 19494123
- Danuser, G. Testing the lamella hypothesis: the next steps on the agenda. J Cell Sci 122, 1959-62 (2009). Pubmed abstract # 19494124
- Chrzanowska-Wodnicka, M. & Burridge, K. Rho-stimulated contractility drives the formation of stress fibers and focal adhesions. J Cell Biol 133, 1403-15 (1996). Pubmed abstract # 8682874
- Hotulainen, P. & Lappalainen, P. Stress fibers are generated by two distinct actin assembly mechanisms in motile cells. J Cell Biol 173, 383-94 (2006). Pubmed abstract # 16651381
- Yarmola, E. G. & Bubb, M. R. Profilin: emerging concepts and lingering misconceptions. Trends Biochem Sci 31, 197-205 (2006). Pubmed abstract # 16542844
- Le Clainche, C. & Carlier, M.-F. Regulation of actin assembly associated with protrusion and adhesion in cell migration. Physiol. Rev. 88, 489-513 (2008). Pubmed abstract # 18391171
- Kislauskis, E. H., Zhu, X. & Singer, R. H. beta-Actin messenger RNA localization and protein synthesis augment cell motility. J Cell Biol 136, 1263-70 (1997). Pubmed abstract # 9087442
- Condeelis, J. How is actin polymerization nucleated in vivo? Trends Cell Biol 11, 288-93 (2001). Pubmed abstract # 11413039
- Bamburg, J. R. & Bernstein, B. W. ADF/cofilin. Curr Biol 18, R273-5 (2008). Pubmed abstract # 18397729
- Maciver, S. K. & Hussey, P. J. The ADF/cofilin family: actin-remodeling proteins. Genome Biol 3, reviews3007 (2002).Pubmed abstract # 12049672
- Cai, L., Marshall, T. W., Uetrecht, A. C., Schafer, D. A. & Bear, J. E. Coronin 1B coordinates Arp2/3 complex and cofilin activities at the leading edge. Cell 128, 915-29 (2007). Pubmed abstract # 17350576
- Uetrecht, A. C. & Bear, J. E. Coronins: the return of the crown. Trends Cell Biol 16, 421-6 (2006). Pubmed abstract # 16806932
- Campellone, K. G. & Welch, M. D. A nucleator arms race: cellular control of actin assembly. Nat Rev Mol Cell Biol 11, 237-51 (2010). Pubmed abstract # 20237478
- Paul, A. S. & Pollard, T. D. Review of the mechanism of processive actin filament elongation by formins. Cell Motil Cytoskeleton 66, 606-17 (2009). Pubmed abstract # 19459187
- Chesarone, M. A., DuPage, A. G. & Goode, B. L. Unleashing formins to remodel the actin and microtubule cytoskeletons. Nat Rev Mol Cell Biol 11, 62-74 (2010). Pubmed abstract # 19997130
- Bear, J. E. & Gertler, F. B. Ena/VASP: towards resolving a pointed controversy at the barbed end. J Cell Sci 122, 1947-53 (2009). Pubmed abstract # 19494122
- Bear, J. E. et al. Antagonism between Ena/VASP proteins and actin filament capping regulates fibroblast motility. Cell 109, 509-21 (2002). Pubmed abstract # 12086607
- Cooper, J. A. & Schafer, D. A. Control of actin assembly and disassembly at filament ends. Curr Opin Cell Biol 12, 97-103 (2000). Pubmed abstract # 10679358
- Zigmond, S. H. Beginning and ending an actin filament: control at the barbed end. Curr Top Dev Biol 63, 145-88 (2004).Pubmed abstract # 15536016
- Mullins, R. D., Heuser, J. A. & Pollard, T. D. The interaction of Arp2/3 complex with actin: nucleation, high affinity pointed end capping, and formation of branching networks of filaments. Proc Natl Acad Sci U S A 95, 6181-6 (1998). Pubmed abstract # 9600938
- Thrasher, A. J. & Burns, S. O. WASP: a key immunological multitasker. Nat Rev Immunol 10, 182-92 (2010). Pubmed abstract # 20182458
- Nolen, B. J. & Pollard, T. D. Structure and biochemical properties of fission yeast Arp2/3 complex lacking the Arp2 subunit. J Biol Chem 283, 26490-8 (2008). Pubmed abstract # 18640983
- Pollitt, A. Y. & Insall, R. H. WASP and SCAR/WAVE proteins: the drivers of actin assembly. J Cell Sci 122, 2575-8 (2009).Pubmed abstract # 19625501
- Vartiainen, M. K. & Machesky, L. M. The WASP-Arp2/3 pathway: genetic insights. Curr Opin Cell Biol 16, 174-81 (2004).Pubmed abstract # 15196561
- Yang, C. et al. Novel roles of formin mDia2 in lamellipodia and filopodia formation in motile cells. PLoS Biol 5, e317 (2007). Pubmed abstract # 18044991
- Insall, R. H. & Machesky, L. M. Actin dynamics at the leading edge: from simple machinery to complex networks. Dev Cell 17, 310-22 (2009). Pubmed abstract # 19758556
- Vicente-Manzanares, M., Ma, X., Adelstein, R. S. & Horwitz, A. R. Non-muscle myosin II takes centre stage in cell adhesion and migration. Nat Rev Mol Cell Biol 10, 778-90 (2009). Pubmed abstract # 19851336
- Palamidessi, A. et al. Endocytic trafficking of Rac is required for the spatial restriction of signaling in cell migration. Cell 134, 135-47 (2008). Pubmed abstract # 18614017
- Schmoranzer, J., Kreitzer, G. & Simon, S. M. Migrating fibroblasts perform polarized, microtubule-dependent exocytosis towards the leading edge. J Cell Sci 116, 4513-9 (2003). Pubmed abstract # 14576345
- Bravo-Cordero, J. J. et al. MT1-MMP proinvasive activity is regulated by a novel Rab8-dependent exocytic pathway. Embo J 26, 1499-510 (2007). Pubmed abstract # 17332756
- Caswell, P. T., Vadrevu, S. & Norman, J. C. Integrins: masters and slaves of endocytic transport. Nat Rev Mol Cell Biol 10, 843-53 (2009). Pubmed abstract # 19904298
- Ezratty, E. J., Bertaux, C., Marcantonio, E. E. & Gundersen, G. G. Clathrin mediates integrin endocytosis for focal adhesion disassembly in migrating cells. J Cell Biol 187, 733-47 (2009). Pubmed abstract # 19951918
- Charras, G. & Paluch, E. Blebs lead the way: how to migrate without lamellipodia. Nat Rev Mol Cell Biol 9, 730-6 (2008).Pubmed abstract # 18628785
- Fackler, O. T. & Grosse, R. Cell motility through plasma membrane blebbing. J Cell Biol 181, 879-84 (2008). Pubmed abstract # 18541702
- Friedl, P. & Wolf, K. Plasticity of cell migration: a multiscale tuning model. J Cell Biol 188, 11-9 (2010). Pubmed abstract # 19951899